Serviços Personalizados
Journal
Artigo
Indicadores
-
Citado por SciELO
-
Acessos
Links relacionados
-
Similares em SciELO
Compartilhar
Portugaliae Electrochimica Acta
versão impressa ISSN 0872-1904
Port. Electrochim. Acta vol.29 no.4 Coimbra 2011
Inhibition of Copper Corrosion in 1 M Nitric Acid - Electro Analytical and Theoretical Study with (E)-(4-(4-Methoxybenzylideneamino)-4H-1, 2, 4-Triazole-3, 5diyl) Dimethanol (MBATD)
Bincy Joseph and Abraham Joseph*
Department of Chemistry, University of Calicut, P.O, 673635, Kerala, India
Abstract
TThe inhibition of copper corrosion in 1 M HNO3 by (E)-(4-(4methoxybenzylideneamino)- 4H-1, 2, 4-triazole-3, 5-diyl) dimethanol (MBATD) was investigated by polarization, ac impedance, adsorption studies, quantum chemical calculations, molecular dynamics methods and surface morphological studies. Polarization studies showed that MBATD acts as a mixed type inhibitor. Adsorption followed the Langmuir isotherm with negative value of free energy. Various thermodynamic and kinetic parameters were calculated to understand the energy changes associated during the inhibition process. The correlation between inhibitive effect and molecular structure is ascertained by quantum chemical calculations using density functional theory and Fukui functions. The molecular dynamics method has also been used to simulate the adsorption of inhibitor molecule on the metal surface.
Keywords: copper, acid solutions, EIS, polarization, acid inhibition.
Introduction
Copper metal plays vital roles in chemical and microelectronic fields due to its high thermal conductivity, electrical conductivity and low cost. It requires strong oxidants for corrosion or dissolution. Nitric acid is one of the most widely used corrosive medium that attracted a great deal of research on copper corrosion [1, 2]. One of the most practical and popular methods for protecting metals against corrosion is the use of inhibitors. Most of the acid corrosion inhibitors are nitrogen, sulphur and or oxygen containing organic compounds. Among these compounds, N-based heterocyclic compounds are found to be efficient inhibitors for copper in acid solutions [3-7].
Quantum-chemical calculations have been found to be quite useful to investigate the reaction mechanisms of the inhibitor molecule and its electronic states [8, 9]. The geometry of the inhibitor molecule in its ground state, nature of its molecular orbital like highest occupied molecular orbital (HOMO) and lowest unoccupied molecular orbital (LUMO), are involved in the inhibition activity. Through DFT calculations it is possible to correlate inhibition property with ionization potential (I), electron affinity (A) and number of electrons transferred (ΔN). Molecular dynamics simulation studies unveil the mechanism of corrosion inhibition through interaction of molecules with surface metal atoms [10-13]. This paper describes the corrosion inhibition efficiency of (E)-(4-(4methoxybenzylideneamino)- 4H-1, 2, 4-triazole-3, 5-diyl) dimethanol (MBATD) towards copper in 1 M nitric acid at various concentrations (25, 50, 100 and 200 ppm) and temperatures (303-323 K) using polarization, electrochemical impedance spectroscopy (EIS), quantum chemical calculations, adsorption studies and surface morphological studies. The thermodynamic functions and activation parameters for the adsorption process were calculated and discussed. In addition, an attempt has also been made to investigate the adsorption of MBATD molecule on the copper (110) surface using molecular dynamics simulations.
Experimental
Inhibitor
The method adopted for the preparation of 1, 2, 4-triazole precursor, 4-amino4H- 1, 2, 4-triazole-3, 5-dimethanol (ATD) is the condensation of glycolic acid with hydrazine hydrate. Hydrazine monohydrate (E. Merck) (3.75 g, 0.75 mol) was added drop wise 8 °C to 70% aqueous glycolic acid (E. Merck) (54.3 g,v 0.50 mol). The resulting solution was heated at 120 °C for 6 h. Then the reflux condenser was replaced with a downward condenser and the reaction mixture was heated at 160 °C for a further 18 h allowing excess hydrazine and water to distil off. After cooling, the yellowish crystalline solid obtained was recrystallized from water to give analytically pure ATD. MBATD was prepared by the condensation of ATD with p-methoxy benzaldehyde (E.Merck) (1:1 molar ratio) using alcohol as the solvent [14-16]. The compound is soluble in 1 M HNO3 at room temperature. The structure of the compound is given in Fig 1. (Molecular formula - C12H14N4O3, molecular weight - 262, melting point - 225-230 °C, elemental analysis % found (calcd.): C, 53.99 (54.96); H, 5.01(5.38); N, 20.89 (21.36); and O, 17.86 (18.30).
Figure 1. Structure of the inhibitor molecule (MBATD).
Medium
The medium for the study was prepared from reagent grade HNO3 (Assay 69% Cl, 0.00005%; SO4, 0.0001%; Pb, 0.000005%; Cu, 0.000005%; Ni, 0.000005%; Zn, 0.00001%; Cd, 0.000001%; Fe, 0.00002%; Ca, 0.0001% and As, 0.000001%, E.merk) and double distilled water. All the tests were performed in aerated medium at different temperatures under normal atmospheric pressure.
Materials
The copper electrode was made from 99.99% pure copper (confirmed with EDX spectra) in the form of circular coupons. The copper specimen was embedded in a Teflon holder with an exposed area of 1 cm2. Before each experiment, the coupons were polished using different grade emery papers followed by washing with different solvents as recommended by ASTM and finally with distilled water.
Electrochemical measurements
Electrochemical tests were carried out in a conventional three-electrode polymethylmethacrylate (PMMA, room temperature)/borosilicate glass (high temperature) cell with platinum (1 cm2 surface area) as auxiliary electrode, and saturated calomel electrode (SCE) as the reference electrode. The working electrode was first immersed in the test solution for establishing a steady state open circuit potential (OCP), and then the electrochemical measurements were carried out with a computer controlled Gill AC electrochemical workstation (ACM, U.K, model no: 1475). Electrochemical impedance spectroscopy (EIS) measurements were carried out in the frequency range 10 kHz to 0.1 Hz with the amplitude of 5 mV (RMS) using a.c signals at open circuit potential. The potentiodynamic polarization studies were performed by sweeping the potential between -250 mV and +250 mV at a scan rate of 1000 mV/ minutes (16 mV/sec). In order to investigate the effects of temperature on the inhibition performance, polarization and impedance studies were carried at various temperatures (303-323 K) in the absence and presence of different concentrations of MBATD (25, 50, 100 and 200 ppm). All electrochemical measurements were made after 1 h immersion in corrosive media in the absence and presence of inhibitors and the reasonable reproducibility is achieved through repetition.
Quantum chemical calculation
Quantum chemical calculations and geometry optimizations were performed using ab initio calculations and the density functional theory (DFT). Ab initio calculations were carried out using the Hatree-Fock (HF) method. The Becke's three-parameter hybrid functional combined with the Lee, Yang and Parr correlation function (B3LYP) was employed in the DFT calculations using 631G (d) basis set. All the quantum chemical calculations were carried out with complete geometry optimization using Gaussian-03W programme package [1719].
The chemical reactivity of different sites of the molecule was evaluated by Fukui indices, defined by:

where qN, qN-1 and qN+1 are the electronic population of the atom k in neutral, cationic and anionic systems, respectively [20, 21]. The condensed Fukui function is to the local reactivity descriptor and thus can be used only for comparing reactive atomic centers within the same molecule, whereas condensed softness indices allow the comparison of the reactivity between similar atoms of different molecules, condensed softness indices which can be calculated easily starting from the relation between the Fukui function ∫(r) and the local softness S(r).

As shown by De Profit et al. [22, 23], the B3LYP functional appears to be reliable for calculating ∫(r) and ∫k indices. The molecular dynamics simulations were performed to understand the adsorption behavior of MBATD on copper (110) surface using Material Studio 4.3 programme package. The copper substrate with (110) plane was first optimized to minimum energy, and then the addition of the MBATD molecule on the Cu (110) surface was simulated using the compass force field [24].
Scanning electron microscopy (SEM)
The surface morphologies of the samples in the absence and presence of the inhibitor were carried out using a digital Scanning Electron Microscope model SU6600 (Serial No: HI-2102-0003) with an accelerating voltage of 20.0 kV, at a scan speed~Slow5 and calibration scan speed of 25. Samples were attached on the top of an aluminium stopper by means of carbon conductive adhesive tape. All micrographs of the specimen were taken at the magnification of 500 xs. These samples underwent the same pre-treatment as used in electrochemical experiments before recording the SEM image.
Results and discussion
Potentiodynamic polarization studies
Tafel polarization curves for copper in unstirred 1 M HNO3 solution in the absence and presence of various concentrations of MBATD at 303, 313 and 323 K are presented in Figs. 2-4. According to corrosion theories [25, 26], the rightward shift of the cathodic curves reveals that the corrosion is mainly accelerated by cathodic reactions. Being a strong oxidizing agent, HNO3 is capable of attacking copper. The Tafel polarization curves exhibit no steep slope in the anodic range, which proves that no passive film is formed on the copper surface. As a result, copper may directly dissolve in 1 M HNO3 solution. Copper is corroded to Cu2+ in HNO3 solutions, and no oxide film is formed to protect the surface from the attack of the corrosive medium. Copper dissolution is thus expected to be the dominant reaction in HNO3 solution. The electrochemical reactions for copper in 1 M HNO3 solution may be described as follows:
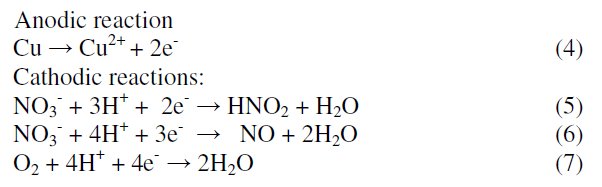
Figure 2. Polarization curve of copper in 1 M HNO3 in the absence and presence of different concentrations of MBATD at 303 K.
Figure 3. Polarization curve of copper in 1 M HNO3 in the absence and presence of different concentrations of MBATD at 313 K.
Figure 4. Polarization curve of copper in 1 M HNO3 in the absence and presence of different concentrations of MBATD at 323 K.
Electrochemical potentiokinetic parameters like corrosion potential (Ecorr), cathodic and anodic Tafel slopes (βc and βa), and corrosion current density (icorr) obtained from Tafel extrapolation of the polarization curve at 303, 313 and 323 K are given in Table 1. The corrosion inhibition efficiency was calculated using the relation:

where i0corr and icorr are uninhibited and inhibited corrosion current density, respectively. It is evident from Figs. 2-4 that the inhibitor molecule reduces both the anodic and cathodic current densities during its inhibitory action. The Tafel slope values given in Table 1 also support this observation. The change in the slopes of the cathodic Tafel lines (βc) and anodic Tafel lines (βa) observed with the addition of the inhibitor molecule is a clear evidence of the action of the inhibitor molecule on cathodic and anodic reactions. These changes in the values of the anodic and cathodic Tafel slopes (βa& βc) suggest that MBATD acts as a mixed type inhibitor. The linear polarization resistance (LPR) obtained from linear polarization curves (another form of Tafel plots, graphs not given) is also shown in Table 1, and it means good inhibition efficiency for MBATD. The presence of defects on the metal surface permits free access to H+ ions and encourages the dissolution process of the metal [27, 28]. The surface coverage increases with the inhibitor concentration. This film reduces the active surface area available to the corrosive medium and delays the gas evolution and copper dissolution [29].
Table 1. Electrochemical parameters for copper obtained from polarization curves in 1 M HNO3 at 303, 313 and 323 K.
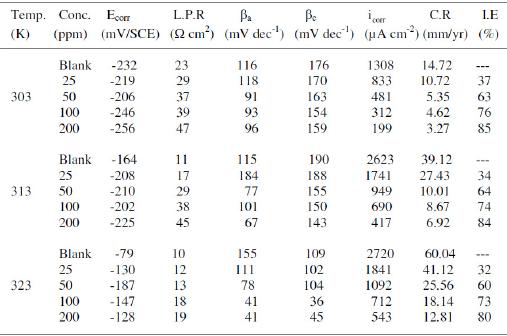
Electrochemical impedance spectroscopy
In order to understand the adsorption and inhibition mechanism of MBATD on copper surface, electrochemical impedance spectroscopy measurements were performed to the copper/electrolyte solution interface. The Nyquist plots and the representative Bode diagrams for the uninhibited 1 M HNO3 and solution containing different inhibitor concentrations at various temperatures are given in Figs. 5-10.
Figure 5. Nyquist plots of copper in 1 M HNO3 in the absence and presence of different concentrations of MBATD at 303 K.
Figure 6. Nyquist plots of copper in 1 M HNO3 in the absence and presence of different concentrations of MBATD at 313 K.
Figure 7. Nyquist plots of copper in 1 M HNO3 in the absence and presence of different concentrations of MBATD at 323 K.
Figure 8. Bode plots of copper in 1 M HNO3 in the absence and presence of different concentrations of MBATD at 303 K.
Figure 9. Bode plots of copper in 1 M HNO3 in the absence and presence of different concentrations of MBATD at 313 K.
Figure 10. Bode plots of copper in 1 M HNO3 in the absence and presence of different concentrations of MBATD at 323 K.
The Nyquist plots display a capacitive loop at high frequencies (may be due to charge transfer of the corrosion process) and a straight line at low frequencies (may be due to diffusion of soluble reactant or product species). It is evident from Figs. 5-7 that the impedance loops measured are depressed semi-circles with their centers below the real axis. This "dispersing effect" is mainly due to the roughness and other in- homogeneities of the electrode surface [30-34]. So, one constant phase element (Q) is substituted for the capacitive element in the equation to get a more accurate fit.
The impedance of a constant phase element is described by the expression:

where Y0 is a proportional factor, n has the meaning of a phase shift. For n = 0, Q represents a resistance, for n = 1, a capacitance, for n = 0.5, a Warburg element and for n =-1 an inductance. According to Hsu and Mansfeld [35], the values of the double layer capacitance (Cdl) can be obtained from the equation:

where ω"m is the frequency at which the imaginary part of the impedance has a maximum.
Charge transfer resistance (Rct) and double layer capacitance (Cdl) are given in Table 2.
Table 2. AC impedance data of copper with MBATD in 1 M HNO3 solutions at 303, 313 and 323 K.
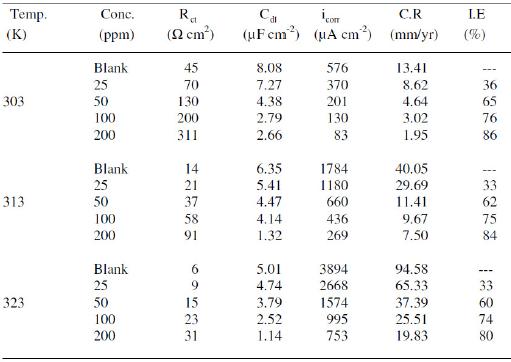
It is observed that the values of the polarization resistance increase with the inhibitor concentration. In the case of impedance studies, %IE is calculated with the equation:

where R0ct and Rct are the values of the charge transfer resistance observed in the absence and presence of MBATD. The inhibition efficiencies calculated from EIS studies show the same trend of polarization measurements. The small difference in the inhibition efficiency of these two methods may be attributed to the different surface status of the electrode in these two measurements. EIS measurements were performed at the rest potential, while in polarization measurements the electrode surface was polarized due to high over potential, non-uniform current distributions resulted from cell geometry, solution conductivity, counter and reference electrode placement, etc., and will lead to the difference between the electrode area actually undergoing polarization and the total area [36]. As it can be seen from Table 2, the Cdl values tend to decrease with the increase of the inhibitor concentration. The decrease in the Cdl, which can result from a decrease in local dielectric constant and/or an increase in the thickness of the electrical double layer, suggests that MBATD molecule functions by adsorption at the metal/solution interface [37].
Adsorption isotherm and thermodynamic parameters
The first step in the inhibition of metallic corrosion is the adsorption of the organic molecule at the metal/solution interface. It depends on the molecule's chemical composition, the temperature, and the electrochemical potential at the metal/solution interface. In fact, the solvent H2O molecules could also adsorb at the metal/solution interface. Thus the adsorption of inhibitor molecule from the aqueous solution can be considered as a quasi-substitution process between the organic compounds in the aqueous phase [Org(sol)] and water molecules at the electrode surface [H2O(ads)] [38].

where x is the size ratio (number of water molecules replaced by one molecule of the organic inhibitor).
Basic information on the interaction between the inhibitor molecule and the copper surface can be obtained from the adsorption isotherm. In order to obtain the isotherm, linear relation between surface coverage (θ) values (θ = % IE /100; Table 2) and inhibitor concentration (Cinh) must be found. From attempts to fit the θ values to different isotherms, the best-fit result is obtained with the Langmuir isotherm.

where Kads is the equilibrium constant of the adsorption process. The plot of (Cinh/θ) versus Cinh gave a straight line at various temperatures (Fig.11). The values of the linear regression coefficients (R2) confirm that the adsorption of MBATD follows Langmuir adsorption isotherm. The free energy of adsorption (ΔGads°) is related to the adsorption constant (Kads) with the following equation [39].

where R is the universal gas constant, T is the thermodynamic temperature and 55.5 is the concentration of water in the solution expressed in mol-1. The values of free energy of adsorption and adsorption constant are listed in Table 3.
Figure 11. Langmuir adsorption isotherm for MBATD on copper surface in 1 M HNO3: 1) at 303 K; 2) at 313 K and 3) at 323 K.
Table 3. Thermodynamic parameters for the adsorption of MBATD in 1 M HNO3 on copper in the presence of 200 ppm at different temperatures.
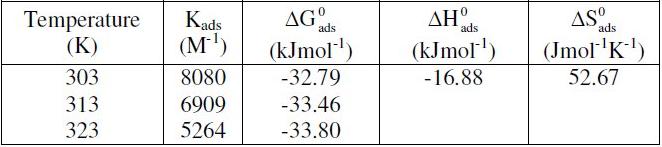
The negative values of ΔG ads ο and the higher values of Kads (≥100M-1) ensure the spontaneity of the adsorption process and are characteristic of strong interaction and stability of the adsorbed layer [40]. However, the value of entropy of adsorption is positive. In fact, it is well known that adsorption is an exothermic phenomenon accompanied by a decrease in entropy. In aqueous solution, the adsorption of organic molecule is generally accompanied with desorption of water molecules. The adsorption of an organic adsorbate at the metal/solution interface is considered as "substitutional adsorption" phenomenon. Therefore, the electrostatic interaction between the charged molecules and the charged metal (physisorption), and the values around -40 kJ/mol or higher are associated with chemisorption, because of sharing or transfer of electrons from organic molecules to the metal surface to form a coordinate type of bond [41]. The value of calculated ΔG ads ο corresponding to MBATD lies between -32 kjmol-1 and -33 kjmol-1. This indicates that the adsorption is not a simple physical adsorption but it may involve some other interactions also. The thermodynamic parameters are also important for studying the mechanism of corrosion inhibition. The heat of adsorption ( ΔH0 ) is calculated using the Van't Hoff equation [42]

To calculate the heat of adsorption, ln Kads and 1/T were plotted and are given in Fig. 12. From the straight line graph the slope (-ΔHads0 /R) and intercept (ΔSads0 /R+ln(1/55.5)) values are calculated. The calculated values of heat of adsorption and entropy of adsorption are given in Table 3. The negative sign of ΔHads0 indicates that the adsorption of the inhibitor molecule is an exothermic process. The value of ΔSads0 in the presence of the inhibitor is positive meaning that an increase in disordering takes place on going from reactants to the metal adsorbed reaction species [43].
Figure 12. Adsorption isotherm plot for ln kads vs. 1/T of MBATD on mild steel in1 M HCl.
Effect of temperature and kinetic parameters
The effect of temperature on various corrosion parameters like Ecorr, icorr and % IE were studied in 1 M HNO3 at three different temperatures, 303, 313 and 323 K, in the absence and presence of different inhibitor concentrations. Variation of temperature has almost no effect on the general shape of the polarization and impedance graphs. The results are listed in Tables 1 and 2. Inspection of these tables shows that, as the temperature increases, the values of icorr increase, and inhibition efficiency and surface coverage decrease. This proves that the inhibition occurs through the adsorption of MBATD on copper surface. The inhibition properties of MBATD can also be explained by kinetic model. The activation parameters were calculated from Arrhenius equation and transition state equations [44, 45]:
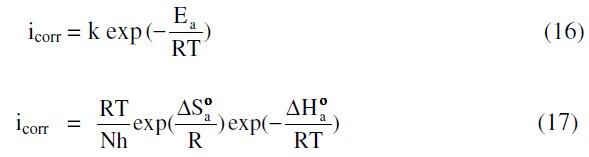
where Ea is the activation energy, k is the Arrhenius pre-exponential factor, T is the absolute temperature, R is the gas constant, h is Planck's constant, N is Avogadro's number, ΔSa0 is the entropy of activation, and ΔHa0 is the enthalpy of activation.
A plot of ln icorr versus 1/T gave a straight line, as shown in Fig. 13. The values of activation energy obtained from the slope of the lines are listed in Table 4. The increase of Ea value with MBATD may be due to either physical adsorption that occurs in the first stage or to the decrease in the adsorption of the inhibitor molecule on the metal surface with increase of temperature [46-49]. To obtain the values of enthalpy and entropy of activation processes, a plot of ln (icorr/T) against 1/T is constructed and given in Figure 14. Straight lines were obtained with a slope of (-ΔH/R) and an intercept of [ln (R/Nh) + (ΔS/R)] and the calculated values of ΔH and ΔS are given in Table 4. The positive signs of the enthalpies (ΔH) reflect the endothermic nature of the metal dissolution process suggesting that the dissolution of copper is slow in the presence of the inhibitor [50].
Figure 13. Arrhenius plots of ln(icorr) versus 1/T: (1) 1 M HCl, (2) 1 M HCl + MBATD.
Table 4. Activation parameters for copper in 1 M HNO3 in absence and presence of MBATD.
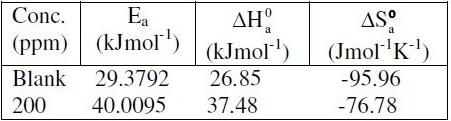
Figure 14. Arrhenius plots of ln(icorr/T) versus 1/T: (1) 1 M HCl, (2) 1 M HCl + MBATD.
Quantum chemical calculations
Quantum chemical calculations are performed to understand the effect of structural parameters on inhibition efficiency of MBATD and to study its adsorption mechanism on copper surface. The optimized structure of MBATD in its ground state is given in Fig.15 (a).
Figure 15. (a) Optimized geometry of the inhibitor molecule, (b) highest occupied molecular orbital (HOMO) of the inhibitor, (c) lowest unoccupied molecular orbital (LUMO) of the inhibitor, and (d) mode of adsorption of the inhibitor molecule on copper (110) plane.
Frontier orbital theory is useful in predicting the adsorption centers of the inhibitor molecule responsible for the interaction with surface metal atoms. It is reported that excellent corrosion inhibitors are usually those organic compounds which are not only offering electrons to unoccupied molecular orbital of the metal, but also accept free electrons from the metal. From the literature it is clear that the higher the HOMO energy of the inhibitor, the greater the trend of offering electrons to the unoccupied 'd' orbital of the metal, and higher will be the corrosion inhibition efficiency. The lower the LUMO energy, the easier the acceptance of electrons by the π orbitals of MBATD from the metal. The decrease in the LUMO-HOMO energy gap increases the efficiency of the inhibitor. The pictorial representations of the HOMO and LUMO of MBATD are given in Fig.15 (b) and (c). Quantum chemical parameters listed in Table 5 reveal that MBATD has high HOMO and low LUMO with low-energy gap and thus possesses higher inhibition efficiency.
Table 5. Various quantum chemical parameters calculated by computational/simulation studies.
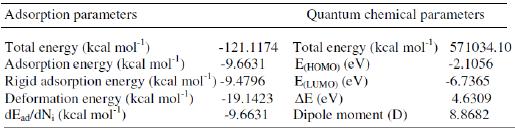
The number of electrons transferred (ΔN) was also calculated using the quantum chemical method by the following equation:

where χCu and χinh denote the absolute electro negativity of copper and the inhibitor molecule, and ηCu and ηinh denote the absolute hardness of copper and inhibitor molecule respectively. These are related to electron affinity (A) and ionization potential (I).

Using a theoretical χ value of 4.48 eV/mol according to Pearson's electro negativity scale, and η value of 0 eV/mol for copper, ΔN was calculated. Values of ΔN show that inhibition effect resulted from electron donation (Table 6). From Lukovits' study, one can derive the conclusion that if ΔN < 3.6, the inhibition efficiency increases with increasing electron-donating ability at the metal surface. Here, MBATD was the donor of electrons, and the copper oxide surface was the acceptor. MBATD was bound to the copper oxide surface, and thus forming inhibitive adsorption layer against corrosion [51-56].
Table 6. Quantum chemical descriptors for MBATD.

Fukui function and chemical reactivity
Fukui indices are used for predicting the preferential site of electrophilic attack at MBATD. They are widely used as descriptors of site selectivity for the soft-soft reactions. According to Li and Evans, the favorite reactive site is that which possesses high value of Fukui indices. For nucleophilic attack the most reactive site of MBATD is the C (17) atom, and for electrophilic attack the most reactive site is the N (16) atom. These results are given in Table 7.The condensed local softness indices Sk- and Sk+ are related to the condensed Fukui functions. The local softness follows the same trend of Fukui functions [57-59].
Table 7. Fukui functions and local softness values for MBATD.
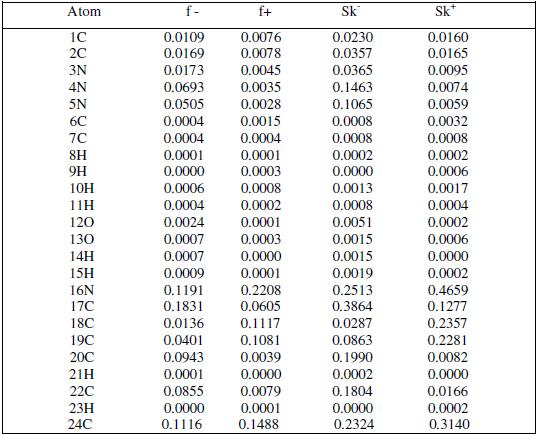
Molecular dynamics simulations
Molecular dynamics simulation studies were performed for understanding the nature of interaction between MBATD and copper surface. Structure of MBATD shows that the molecule is likely to adsorb on the metal surface by sharing electron of nitrogen atom, phenyl ring and triazole structure. The periodic boundary conditions were applied to the simulation cell and the whole system was energy optimized, and the possibility of the MBATD adsorption on the copper surface was simulated as in Fig. 15(d). The adsorption energy, the sum of the rigid adsorption energy and deformation energy for the adsorbate components have been calculated. The rigid adsorption energy reports the energy released in kcal/mol, when the unrelaxed adsorbate components adsorbed on the substrate. The deformation energy reports the energy released when the adsorbed adsorbate components are relaxed on the substrate surface. The total energy, rigid adsorption energy, deformation energy and dEad/dNi were calculated by the Monte Carlo simulation [10, 60, and 61] for the adsorption of MBATD molecule on Cu (110) plane and are presented in Table 5. These findings also confirm the adsorption of MBATD on copper surface.
Scanning electron microscopy
SEM was employed to explore additional information on the inhibition mechanism. The SEM micrographs of copper metal before and after exposure in 1 M HNO3 are shown in Fig. 16(a) and (b). These pictures clearly show that the surface was covered with high-density pits due to the exposure of copper in nitric acid. The influence of the addition of 200 ppm MBATD on copper metal in 1 M HNO3 is shown in Fig.16(c). The micrograph shows no evidence of pitting. This may be due to the adsorption of inhibitor molecules around the pits formed on the specimens in the early stages of formation (initiation and propagation).
Figure 16. SEM micrographs of (a) copper metal before immersion in 1 M HNO3; (b) after immersion in 1 M HNO3, and (c) after immersion in 1 M HNO3 containing inhibitor molecule.
Conclusions
1) The inhibitor molecule shows very high inhibitive efficiency for copper in 1 M HNO3.
2) The percentage inhibition efficiency increases with increase in concentration and decreases with temperature.
3) The adsorption of MBATD on copper surface follows Langmuir adsorption isotherm. The inhibitor molecules adsorb on the copper surface blocking the reaction sites. The surface area available for the attack decreases with increasing inhibitor concentrations.
4) Polarization studies reveal that MBATD acts as a mixed type inhibitor.
5) The corrosion inhibition efficiency of MBATD and the values EHOMO, ELUMO, ELUMO-EHOMO and ΔN of MBATD, calculated by DFT method, are correlated.
6) The molecular dynamics stimulation results reveal the adsorption of MBATD molecule on the copper surface.
References
1. A. Fiala, A. Chibani, A. Darchen, A. Boulkamh, K. Djebbar, Appl. Surf. Sci. 253 (2007) 9347. [ Links ]
2. K.F. Khaled, Corros. Sci. 52 (2010) 3225. [ Links ]
3. F. Zucchi, G. Trabanelli, C. Monticelli, Corros. Sci. 38 (1996) 147. [ Links ]
4. S.A. Abd El-Maksoud, h.H. Hassan, Mater. Corros. 58 (2007) 5. [ Links ]
5. G. Singh, J. Telegad, E. Kalman, Paper 331 in 13th International Corrosion Congress, Melbourne, Australia (1996). [ Links ]
6. F .Mansfeld, Y. Wang, Paper Nº 41, in Corrosion'95 NACE (1995). [ Links ]
7. A.J. McMahon, D. Harrop, Paper Nº 32 in Corrosion'95. NACE (1995). [ Links ]
8. C. Adamo, V. Barone, Chem. Phys. Lett. 330 (2000) 152. [ Links ]
9. M. Parac, S. Grimme, J. Phys. Chem. A 10 (2003) 6844. [ Links ]
10. K.F. Khaled, Electrochim. Acta 53 (2008) 3484. [ Links ]
11. K.F. Khaled, Electrochim. Acta 54 (2009) 4345. [ Links ]
12. K.F. Khaled, M.A. Amin, Corros. Sci. 51 (2009)1964. [ Links ]
13. Y. Tang, X. Yang, W. Yang, Y. Chen, Rong Wan, Corros. Sci. 52 (2010) 242.
14. M. Adamek, Coll. Czech. Chem. Commun. 25 (1960) 1694. [ Links ]
15. W. Vreugdenhil, J.G. Haasnoot, J. Reedijk, A.L. Spek, Inorg. Chim. Acta 129 (1987) 205. [ Links ]
16. P.J. van Koningsbruggen, J.W. van Hal, R.A.G. de Graff, JG. Haasnoot, J. Reedijk, J. Chem. Soc. Dalton Trans. (1993) 2163. [ Links ]
17. M.J. Frisch, G.W. Trucks, H.B. Schlegel et all, Gaussian 03, Revision B.05. Gaussian Inc., Pittsburg.(2003). [ Links ]
18. N. Lopez, F. Illas, J. Phys. Chem. B 102 (1998) 1430. [ Links ]
19. C. Lee, W. Yang, R.G. Parr, Phys. Rev. B 37 (1988) 785. [ Links ]
20. R.G. Parr, W. Yang, "DFT of atoms and molecules", Oxford University Press, Oxford (1989). [ Links ]
21. A. E. Reed, L.A. Curtiss, F. Weinhold, Chem. Rev. 88 (1988) 899. [ Links ]
22. A.D. Becke, J. Chem. Phys. 98 (1993) 5648. [ Links ]
23. F.de Proft ,J.M.L. Martin, P. Geerlings, Chem. Phys. Lett. 256 (1996) 400. [ Links ]
24. Y.M. Tang, X.Y. Yang, W.Z. Yang, Y. Chen, Rong Wan, Corros. Sci. 52 (2010) 246.
25. D.A. Jones, "Principles and Prevention of Corrosion", 2nd ed., Prentice Hall, NJ (1983). [ Links ]
26. K.F. Khaled, Sahar A. Fadl-Allah, B. Hammouti, Mater. Chem. Phys. 117 (2009) 151.
27. A. Chetouani, B. Hammouti, T. Benhadda, M. Daoudi, App. Surf. Sci. 249 (2005) 375.
28. B. El Mehdi, B. Mernari, M. Traisnel, F. Bentiss, M. Lagrenee, Mater. Chem. Phys. 77 (2002) 489.
29. R. Fuchs-Godec, Colloids Surf. A: Physicochem. Eng. Aspects 280 (2006) 130. [ Links ]
30. O. Benali, L. Larabi, Y. Harek, J. Saudi Chem. Soc. 14 (2010) 233. [ Links ]
31. Da-Quan Zhang, Qi-Rui Cai, Xian-Ming He, Li-Xin Gao, Gui Soon Kim, Mat. Chem. Phys. 114 (2009) 614.
32. F. Mansfeld, Corrosion 37 (1981) 301. [ Links ]
33. E. MacCafferty, Corros. Sci. 39 (1997) 243. [ Links ]
34. M.S. Morad, Corros. Sci. 42 (2000) 1313. [ Links ]
35. C.H. Hsu, F. Mansfeld, Corrosion 57 (2001) 747. [ Links ]
36. R.G. Kelly, J.R. Scully, D.W. Shoesmith, R.G. Buchheit, "Electrochemical Techniques in Corrosion Science and Engineering", Marcel Dekker, Inc., New York (2002). p. 148.
37. M. Lebrini, M. Lagren, M. Traisnel, L. Gengembre, H. Vezin, F. Bentiss, Appl. Surf. Sci. 253 (2007) 9267.
38. M. Sahin, S. Bilgic, H. Yilmaz, Appl. Surf. Sci. 1 (2002) 195. [ Links ]
39. E. Cano, J.L. Polo, A. La Iglesia, J.M. Bastidas, Adsorption 10 (2004) 219.
40. M. Lagrenee, B. Mernari, M. Bouanis, M. Traisnel, F. Bentiss, Corros. Sci. 44 (2002) 73.
41. M. Hosseini, S.F.L. Mertens, M.R. Arshadi, Corros. Sci. 45 (2003) 1473. [ Links ]
42. M.A. Amin, S.S. Abd El Rehim, H.T.M. Abdel-Fatah, Corros. Sci. 51 (2009) 882. [ Links ]
43. M. Bouklah, B. Hammouti, M. Lagrenee, F. Bentiss, Corros. Sci. 48 (2006) 2831.
44. A. Ostovari, S.M. Hoseinieh, M. Peikari, S.R. Shadizadeh, S.J. Hashemi, Corros. Sci. 10 (2009) 1016.
45. L. del Campo, R.B. Perez-Saez, L. Gonzalez-Fernandez, M.J. Tello, Corros. Sci. 51 (2009) 707.
46. E.A. Noor, A.H. Al-Moubaraki, Mater. Chem. Phys. 110 (2008) 145. [ Links ]
47. L. Larabi, Y. Harek, O. Benali, S. Ghalem, Prog. Org. Coat. 54 (2005) 256.
48. L. Larabi, O. Benali, Y. Harek, Mater. Lett. 61 (2007) 3287. [ Links ]
49. T. Szauer, A. Brandt, Electrochim. Acta 26 (2004) 1209. [ Links ]
50. N.M. Guan, L. Xueming, L. Fei, Mater. Chem. Phys. 86 (2004) 59. [ Links ]
51. J. Fang, J. Li, J. Mol. Struct.(THEOCHEM) 593 (2002) 179. [ Links ]
52. G. Bereket, E. Hur, C. Ogretir, J. Mol. Struct. (THEOCHEM) 79 (2002) 578. [ Links ]
53. P. Zhao, Q. Liang, Y. Li, Appl. Surf. Sci. 252 (2005) 1596. [ Links ]
54. R.G. Pearson, Nat. Acad. Sci. 83 (1986) 8440. [ Links ]
55. R.G. Pearson, Inorg. Chem. 27 (1988) 734. [ Links ]
56. I. Lukovits,E. Kalman, F. Zucchi, Corrosion 57 (2001) 3. [ Links ]
57. P.W. Ayers, M. Levy, Theor. Chem. Acta 103 (2000) 353. [ Links ]
58. P. Geerlings, F.De Proft, Int. J. Mol. Sci. 3 (2002) 276. [ Links ]
59. Y. Li, J.N.S. Evans, J. Am. Chem. Soc. 117 (1995) 7756. [ Links ]
60. K.F. Khaled, Appli. Sur. Sci. 255 (2008) 1811-1818. [ Links ]
61. K.F. Khaled, Mohammed A. Amin, J. Appl. Electrochem. 39(12) (2009) 2553.
*Corresponding author. E-mail address drabrahamj@gmail.com
Received 21 February 2011; accepted 14 June 2011
Acknowledgement
The authors are grateful to Kerala State Council for Science Technology and Environment (KSCSTE) for financial support in the form of a major research project 018/SRSPS/2006/CSTE.